Last time we stated and proved a simple version of Fisher’s fundamental theorem of natural selection, which says that under some conditions, the rate of increase of the mean fitness equals the variance of the fitness. But the conditions we gave were very restrictive: namely, that the fitness of each species of replicator is constant, not depending on how many of these replicators there are, or any other replicators.
To broaden the scope of Fisher’s fundamental theorem we need to do one of two things:
1) change the left side of the equation: talk about some other quantity other than rate of change of mean fitness.
2) change the right side of the question: talk about some other quantity than the variance in fitness.
Or we could do both!
People have spent a lot of time generalizing Fisher’s fundamental theorem. I don’t think there are, or should be, any hard rules on what counts as a generalization.
But today we’ll take alternative 1). We’ll show the square of something called the ‘Fisher speed’ always equals the variance in fitness. One nice thing about this result is that we can drop the restrictive condition I mentioned. Another nice thing is that the Fisher speed is a concept from information theory! It’s defined using the Fisher metric on the space of probability distributions.
And yes—that metric is named after the same guy who proved Fisher’s fundamental theorem! So, arguably, Fisher should have proved this generalization of Fisher’s fundamental theorem. But in fact it seems that I was the first to prove it, around February 1st, 2017. Some similar results were already known, and I will discuss those someday. But they’re a bit different.
A good way to think about the Fisher speed is that it’s ‘the rate at which information is being updated’. A population of replicators of different species gives a probability distribution. Like any probability distribution, this has information in it. As the populations of our replicators change, the Fisher speed says the rate at which this information is being updated. So, in simple terms, we’ll show
The square of the rate at which information is updated is equal to the variance in fitness.
This is quite a change from Fisher’s original idea, namely:
The rate of increase of mean fitness is equal to the variance in fitness.
But it has the advantage of always being true… as long the population dynamics are described by the general framework we introduced last time. So let me remind you of the general setup, and then prove the result!
The setup
We start out with population functions
one for each species of replicator
obeying the Lotka–Volterra equation

for some differentiable functions
called fitness functions. The probability of a replicator being in the ith species is

Using the Lotka–Volterra equation we showed last time that these probabilities obey the replicator equation

Here
is short for the whole list of populations
and
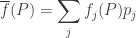
is the mean fitness.
The Fisher metric
The space of probability distributions on the set
is called the (n-1)-simplex

It’s called
because it’s (n-1)-dimensional. When
it looks like the letter 
The Fisher metric is a Riemannian metric on the interior of the (n-1)-simplex. That is, given a point
in the interior of
and two tangent vectors
at this point the Fisher metric gives a number

Here we are describing the tangent vectors
as vectors in
with the property that the sum of their components is zero: that’s what makes them tangent to the (n-1)-simplex. And we’re demanding that
be in the interior of the simplex to avoid dividing by zero, since on the boundary of the simplex we have
for at least one choice of $i.$
If we have a probability distribution
moving around in the interior of the (n-1)-simplex as a function of time, its Fisher speed is

if the derivative
exists. This is the usual formula for the speed of a curve moving in a Riemannian manifold, specialized to the case at hand.
Now we’ve got all the formulas we’ll need to prove the result we want. But for those who don’t already know and love it, it’s worthwhile saying a bit more about the Fisher metric.
The factor of
in the Fisher metric changes the geometry of the simplex so that it becomes round, like a portion of a sphere:
But the reason the Fisher metric is important, I think, is its connection to relative information. Given two probability distributions
the information of
relative to
is
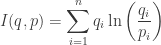
You can show this is the expected amount of information gained if
was your prior distribution and you receive information that causes you to update your prior to
So, sometimes it’s called the information gain. It’s also called relative entropy or—my least favorite, since it sounds so mysterious—the Kullback–Leibler divergence.
Suppose
is a smooth curve in the interior of the (n-1)-simplex. We can ask the rate at which information is gained as time passes. Perhaps surprisingly, a calculation gives
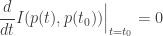
That is, in some sense ‘to first order’ no information is being gained at any moment
However, we have

So, the square of the Fisher speed has a nice interpretation in terms of relative entropy!
For a derivation of these last two equations, see Part 7 of my posts on information geometry. For more on the meaning of relative entropy, see Part 6.
The result
It’s now extremely easy to show what we want, but let me state it formally so all the assumptions are crystal clear.
Theorem. Suppose the functions
obey the Lotka–Volterra equations:

for some differentiable functions
called fitness functions. Define probabilities and the mean fitness as above, and define the variance of the fitness by

Then if none of the populations
are zero, the square of the Fisher speed of the probability distribution
is the variance of the fitness:

Proof. The proof is near-instantaneous. We take the square of the Fisher speed:
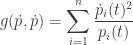
and plug in the replicator equation:

We obtain:

as desired. █
It’s hard to imagine anything simpler than this. We see that given the Lotka–Volterra equation, what causes information to be updated is nothing more and nothing less than variance in fitness!
The whole series:
• Part 1: the obscurity of Fisher’s original paper.
• Part 2: a precise statement of Fisher’s fundamental theorem of natural selection, and conditions under which it holds.
• Part 3: a modified version of the fundamental theorem of natural selection, which holds much more generally.
• Part 4: my paper on the fundamental theorem of natural selection.